Pierre Fongho Suh1,2, Emmanuel Elanga‐Ndille3, Magellan Tchouakui3, Maurice Marcel Sandeu3,4, Darus Tagne1,5, Charles Wondji1,6 and Cyrille Ndo1,7*
Impact of insecticide resistance on malaria vector competence: a literature review
Abstract
Since its first report in Anopheles mosquitoes in 1950s, insecticide resistance has spread very fast to most sub‐Saharan African malaria‐endemic countries, where it is predicted to seriously jeopardize the success of vector control efforts, leading to rebound of disease cases. Supported mainly by four mechanisms (metabolic resistance, target site resist‐ ance, cuticular resistance, and behavioural resistance), this phenomenon is associated with intrinsic changes in the resistant insect vectors that could influence development of invading Plasmodium parasites. A literature review was undertaken using Pubmed database to collect articles evaluating directly or indiretly the impact of insecticide resist‐ ance and the associated mechanisms on key determinants of malaria vector competence including sialome composi‐ tion, anti‐Plasmodium immunity, intestinal commensal microbiota, and mosquito longevity. Globally, the evidence gathered is contradictory even though the insecticide resistant vectors seem to be more permissive to Plasmodium infections. The actual body of knowledge on key factors to vectorial competence, such as the immunity and micro‐ biota communities of the insecticide resistant vector is still very insufficient to definitively infer on the epidemiological importance of these vectors against the susceptible counterparts. More studies are needed to fill important knowl‐ edge gaps that could help predicting malaria epidemiology in a context where the selection and spread of insecti‐ cide resistant vectors is ongoing.
Keywords Plasmodium, Anopheles, Insecticide resistance, Vector competence
Impact of insecticide resistance on malaria vector competence: a literature review
Background
Malaria is the biggest killer among vector-borne dis- eases [1] and has claimed the lives of milllions of peo- ple over centuries [2]. In 2020, 241 million cases were reported leading to 627,000 deaths. The African region has paid the highest tributes with 96% of all deaths [3]. Malaria disease is caused by Plasmodium parasites, which are transmitted to humans by the bites of infected female mosquitoes of the genus Anopheles [4]. In Africa, Plasmodium falciparum is the most epidemiologically important of malaria parasites infecting humans [5], and Anopheles gambiae, Anopheles coluzzii, Anopheles funes- tus and Anopheles arabiensis are the dominant vector species [6].
Malaria control includes medical treatment of cases and protective measures against the vectors to prevent
Impact of insecticide resistance on malaria vector competence: a literature review
and/or limit contacts with human hosts during which transmission occurs. The control of mosquito popula- tions on a large scale using insecticide-treated nets (ITNs) and indoor residual spraying, associated with increase case management, has led to a remarkable reduction in malaria burden from 81.1 cases per 1000 population in 2000 to 58.9 in 2015 [3]. After this period, the impact of control efforts on malaria burden have dwindled, coinciding with the spread of insecticide resistant vec- tors across most endemic countries [3, 7]. Resistance of Anopheles mosquitoes to insecticides, reported for the first time in Africa in the 1950s [7], concerns four main classes of insecticides used in public health for vector control purposes, namely pyrethroids, organochlorines, organophosphates and carbamates [7, 8]. There are four mechanisms deployed by mosquitoes to become insensi- tive to the insecticides, including by order of importance (1) degradation of insecticide molecules by detoxification enzymes (metabolic resistance), (2) modification of the target affinity of the insecticide (target site resistance), (3) reduced penetration of the insecticide (cuticular resist- ance) and, (4) avoidance of insecticide-treated surfaces (behavioural resistance). Of these four mechanisms tar- get site and metabolic resistances are most likely to lead to control failure [9].
In target site resistance, a change (leucine changed to a phenylalanine or a serine at position 1014) occurring in the amino acid sequence of the voltage gate sodium channel (vgsc) leads to a reduced sensitivity of mosqui- toes to pyrethroids and organochlorines. This phenotype is known as knock down resistance or kdr [10, 11]. When the amino acid change (glycine replaced by serine at position 119) occurs in the neurotransmitter acetyl-cho- linesterase, it occasions resistance to organophosphates and carbamates, termed ace-1 resistance [12, 13]. About metabolic resistance, insecticide resistant mosquitoes increase the expression of detoxification enzymes, such as the cytochrome P450 monooxygenases, glutathione S-transferases (GSTs) and esterases, that eliminates xeno- biotic compounds (including insecticides) before they reach their target. In another instance, an amino acid substitutions in the sequence of detoxification enzymes could modifiy its affinity with the insecticides in insect vectors [14]. For example, several cytochrome P450 genes (CYP6P9a, CYP6P9b and CYP6M7) are involved in resistance to pyrethroids in the species An. funestus [15, 16]; while a substitution of leucine by phenylalanine at position 119 in the epsilon class of GST (GST2– L119F) confers a cross-resistance to dichloro-diphenyl-trichloro- ethane (DDT) and pyrethroids in the same vector species [17].
Despite the widespread distribution of insecticide resistance, its impact on overall malaria epidemiology remains unclear and is currently a subject of intense debate. The evaluation of the potential impact of insec- ticide resistance on vectorial competence is therefore becoming an important and urgent research theme whose findings will help understanding whether it alters or enhances the permissiveness of malaria vectors to Plasmodium parasites, from its early stage (ookinete) to the infective form (sporozoite). In this review, the evi- dence of insecticide resistance impact on the infectivity of mosquitoes to Plasmodium was explored in the litera- ture, and changes in intrinsic factors that could predict or explain the outcome of an infectious blood meal intake were broached. Finally, the knowledge gaps were pointed out.
Search strategy
A literature search was undertaken in the PubMed data- base to extract articles addressing the following themes: (1) Plasmodium infection in insecticide resistant malaria vectors, (2) sialome of insecticide resistant malaria vec- tors, (3) effect of insecticide resistance on the immunity of malaria vectors, (4) microbiota of insecticide resist- ant malaria vectors and infection, and (5) fitness cost of insecticide resistance in malaria vectors. The first search terms were “Anopheles” and “insecticide resistance” and they were associated with either “Plasmodium infection”, “vector competence”, “salivary gland”, “sialome”, “micro- biota”, “gene expression” or “longevity”. Additional articles were extracted from the references lists of the full pub- lications. The search was done between February and August 2022 and there was no restriction regarding the date of publication of the articles. A total of 560 articles were obtained from the search. Articles that addressed insecticide resistance in Anopheles in a broad manner, and not in relation with either Plamodium infection, vec- tor competence, sialome, or longevity were discarded. Therefore, 28 articles related to the themes mentioned above were selected and used for the review.
Malaria vector competence
Vector competence is the intrinsic ability of anophe- line species or populations to allow the development of Plasmodium parasites from ookinete to infective sporo- zoites. When a mosquito takes an infectious blood meal from human, the gametocytes ingested begin their development in the midgut. The male gametocyte transform into eight microgametes after three rounds of mitosis, meanwhile the female gametocytes matures into macrogametes [4]. These cells fuse to form zygotes that thereafter change into ookinetes in the lumen of the intestine. The ookinetes then strive through the epithelium of the midgut and once in its basal side, transform into oocysts. The oocysts undergo several rounds of asexual multiplication (sporogony) leading to the production of thousands of haploid sporozoites in each oocyst. Mature occysts rupture and release sporo- zoites in the hemocoel, which immediately migrate to the salivary glands. The extrinsic incubation period of the parasite is about 14 days with the transition from ookinetes to mature oocysts having the highest dura- tion (about 10 days) [18, 19].
In mosquito host, Plasmodium face several immune- related bottlenecks deployed to prevent the success- ful transition from its early stage in the midgut to the sporozoite stage in the salivary glands [18]. The out- come of the parasite infection is reported to depend mainly on the mosquito-Plasmodium genetics adapta- tion [19, 20]. Another very important factor that influ- ences the above outcome is the compatibility of the duration of parasite development with the longevity of the mosquitoes [21, 22]. Only species in which Plas- modium reaches infective form are referred to as com- petent vectors and could ensure malaria transmission. The impact of vector competence on the transmission of malaria can be estimated using Ro (Fig. 1), the basic reproductive number developed by McDonald in 1957. The McDonald model gives the threshold for a disease to persist or spread (Ro greater than 1) or to disappear (Ro less than 1) [23]. The Ro represents the number of individuals in a susceptible human population that are expected to get infected via a mosquito bite when a sin- gle infected individual is present in the population [24, 25]. In the Ro equation, two parameters are related to vector competence: probability of mosquito infection (b)andmosquitolongevity(p)(Fig.1).Modificationsof the values of components of this equation for a given vector population will cause either an augmentation or reduction in the transmission dynamics of the disease, leading probably to a change in the epidemiological profile of the locality concerned. It was established that an increase in b will increase the Ro, whereas a decrease in p will cause the opposite [26].
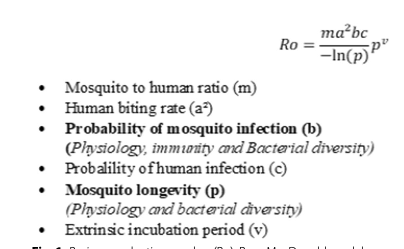
Insecticide resistance and malaria vector infectivity
to Plasmodium parasite
The rapid spread of insecticide resistance among malaria vectors accross endemic countries in the past dec- ade have raised several questions among which that of knowing what is its impact on mosquito permissiveness to Plasmodium? Only a limited number of studies have tried to elucidate this question [27–35]. These stud- ies compared P. falciparum infection rates in resistant Anopheline vectors with susceptible ones, either caught in the field or experimentally infected (Table 1).
Anopheles gambiae strain bearing kdr resistance allele (Vgsc–L1014S) was found naturally more infected by sporozoites than the susceptible counterpart [27]. Similar findings were experimentally observed in the same spe- cies, as well as in Anopheles coluzzii [30, 32]. Contrary to kdr resistance, An. gambiae with ace-1 resistance allele did not differ from individuals that have the wild type allele (not conferring insecticide resistance) on infec- tion rate despite significantly higher oocyst prevalences were observed in the resistant strain [32]. More studies using field populations are needed to ascertain whether a lower longevity suspected by the author and/or other factors are involved.
Regarding metabolic resistance, recent breakthroughs in designing simple PCR-based assays to detect glu- tathione S-transferase (GST)-based and cytochrome P450-mediated resistance in An. funestus sensu stricto provided a unique opportunity to assess its impact on the mosquito’s ability to develop the parasites. The L119F- GSTe2 resistant genotypes of this species showed, in an experimental infection study, higher permissiveness to oocyst infections than susceptible ones [31]. Similarly, in naturally infected populations of the same species, homozygote L119F-GSTe2 genotypes were found more infected by sporozoites though no significant difference was found at the level of oocyst prevalence [28]. In other hands, Lo and Coetzee [36], infecting experimentally two selected sub-colonies of FUMOZ displaying different degree of pyrethroid resistance by Plasmodium berghei, found that the insecticide resistant colonies were less permissive to infection than the susceptible ones. No investigation has so far explored the relationship between P450s genes implicated in insecticide resistance and P. falciparum infection in An. funestus. Moreover, because of the absence of markers of metabolic resistance in An. gambiae sensu lato such studies are still lacking in these species.
Mosquito species | Type of experiment performed | Type of infection | Infection outcome | References | |
Prevalence | Intensity | ||||
An. gambiaea | kdr‐resistant strain compared to susceptible strain | Natural | Higher sporozoite infection prevalence in resistant strains | [27] | |
An. funestus s.sa | GSTe2‐resistant genotypes compared to susceptible genotypes | Natural | No difference in oocysts infec‐ tion prevalence ns Higher sporozoite infection prevalence in resistant strains | [28] | |
An. gambiae s.l.a | ace-1‐resistant strain com‐ pared to susceptible strain | Natural | Higher oocyst infection preva‐ lence in resistant strains | [29] | |
An. gambiae s.l.a | kdr‐resistant strain compared to susceptible strain | Natural | No difference in oocysts infec‐ tion prevalence ns | [29] | |
An. gambiaea | kdr‐resistant strain compared to susceptible strain | Experimental | Higher oocyst infection preva‐ lence in resistant strains Higher sporozoite infection prevalence in resistant strains | Higher oocyst load in the resistant strains Higher sporozoite infection load in the resistant strains | [30] |
An. coluzziia | kdr‐resistant strain compared to susceptible strain | Experimental | Higher oocyst prevalence in the resistant strains Higher sporozoite infection prevalence in resistant strains | Higher oocysts load in the resistant strains Higher sporozoite load preva‐ lence in the resistant strains | [30] |
An. funestus s.sa | GSTe2‐resistant genotypes compared to susceptible genotypes | Experimental | Lower oocyst infection preva‐ lence in homozygous resistant genotypess | Higher oocysts load inhomozygous and heterozy‐ gous resistant genotypess | [31] |
An. gambiaeb | ace-1‐resistant strain com‐ pared to susceptible strain | Experimental | Higher oocyst prevalence infection in resistant strains No difference in sporozoite infection prevalencens | No difference in oocyst and sporozoite infection loadns | [32] |
An. gambiaeb | kdr‐resistant strain compared to susceptible strain | Experimental | Higher oocyst infection preva‐ lence in resistant strains Higher sporozoite infection prevalence in resistant strains | Lower oocyst and sporozoite infection load in resistant strains | [32] |
An. gambiaeb | ace-1‐resistant strain com‐ pared to susceptible strain | Expérimental | Higher oocyst infection preva‐ lence in resistant strains | [33] | |
An. gambiaeb | kdr‐resistant strain compared to susceptible strain | Higher oocyst infection preva‐ lence in resistant strains | [33] | ||
An. gambiaeb | ace-1‐resistant strain com‐ pared to ace-1‐resistant strain exposed to insecticides | Experimental | Lower oocyst infection preva‐ lence in resistant strains | Lower oocyst infection load in resistant strains | [34] |
An. gambiaea | kdr‐resistant strain compared to kdr‐resistant strain exposed to insecticide‐treated nets | Experimental | Lower oocyst infection preva‐ lence in resistant strains | Lower oocyst infection load in resistant strains | [35] |
a: field strain; b: laboratory strain; ns: Non Significant; s: Significant; α: insecticide exposure to confirm resistance status probably post infection; β: insecticide exposure to confirm resistance status prior to infection
Impact of insecticide resistance on mosquito sialome
Bloodsucking arthropods, like mosquitoes, have evolved saliva containing a mixture of pharmacologically active molecules that help them counteract the hemostatis and inflammatory responses of the vertebrate host during bites, thus facilitating blood meal intake [37]. However, the activity of these molecules goes beyond the scope of ensuring blood meal success, as they possibly influ- ence the completion of Plasmodium development in the salivary gland of malaria vectors. Proteins secreted by
the salivary gland belong to several families (D7, mucin, gSG1, gSG2, gSG6 peptide, gSG7, cE5, 8.2-kDa, 6.2-kDa, etc.) [38] whose function include (1) cytoskeletal and structural activities (2) digestion, (3) circadian rythm and chemosensory, (3) immunity, (4) metabolism and other [39]. The development of insecticide resistance in malaria vectors is accompanied by physiological changes [26] that may affect the sialome composition with consequences on the vector competence. Few studies have investigated changes in the sialome in the insecticide resistant vectors [40, 41].
The secretory protein 100 kDa, which is encoded by Saglin (a cytoskeletal and structural gene present in An. gambiae salivary gland) was considered as the binding target of P. falciparum and P. berghei on salivary gland prior to penetration into the latter [42]. This protein was found down-regulated in ace-1 bearing An. gam- biae strain, suggesting an impact on the vector infectiv- ity to Plasmodium [43]. However, a recent study showed that the 100 kDa Protein is unevenly distributed on the salivary glands lobes. Its absence on the primary site of sporozoites occupancy in the salivary glands, the distal lateral lobes, implies that this protein may instead have a secondary role in the infection of the organ [44–46].
The D7 salivary family has been identified in malaria vectors among the most expressed proteins involved in the antihemostatic activity and probably in digestion of blood meal [47–50]. Elanga et al. [40] showed that two short forms of the D7 family genes (D7r3 and D7r4) are over-expressed in pyrethroid resistant An. funestus (L119F–GSTe2), whereas almost all D7 genes are under- expressed in pyrethroid resistant An. gambiae (kdr, L1014F). A comparable observation was made in insec- ticide resistant Culex quinquefasciatus (ace-1 resistance) [51] as well as in two strains of Aedes aegypti (homozy- gotes resistant C1534 and G1016 kdr) [52]. These find- ings show that insecticide resistance mechanism may affect the sialome composition differently.
Several immune proteins such as the anti-microbial peptides cecropin and defensin were found in the saliva of mosquitoes [39, 53]. These immune proteins under- score the role of the salivary gland in the refractoriness of the Anopheles to infections [39, 53]. The small num- ber of studies that evaluated the impact of insecticide resistance alleles on salivary gland gene expression in mosquito vectors have not reported significant changes related to immune genes as compared with the suscepti- ble counterparts [41, 43, 51, 52], alluding that the resist- ant status to insecticide does not influence noticeably the immune component of the sialome. If these factors are indeed unchanged regardless of the mosquito allelic composition, nothing is known whether under infection the expression profile of these immune proteins will vary or not according to the mosquito genotype. Das et al. [39] and Djegbe et al. [51] demonstrated that salivary gland genes expression is influenced by blood meal intake and varies towards the period coinciding with the matura- tion of Plasmodium parasites in mosquitoes [54]. This evidence was not previously studied and should be taken into account in subsequent research works that aims at identifying differentially expressed genes of the salivary
gland and elucidating their impact on the malaria vector competence.
Impact of insecticide resistance on vector immunity
When the infectious blood meal reaches the midgut of the female Anopheles, the immune system is deployed to prevent infections [20]. In the midgut, P. falciparum faces the peritrophic membrane, a physical barrier developed to prevent infections. It also protects against the damag- ing effects of the human blood factors like antibodies and regulates several digestive enzymes [55, 56]. Enzymes such as trypsin 1 and 2, chymotrypsin, carboxypeptidase, aminopeptidase and serine protease are upregulated during digestion to cleave the large content of proteins in the blood meal [57–60]. These proteases are appar- ently involved in the elimination of Plasmodium infec- tions [61]. Three studies attempted to elucidate the effect of insecticide resistance on vectors’ immunity [62–64]. Mitri et al. [62], in a study evaluating genes implicated in the infectivity of An. coluzzii, demonstrated that the kdr– bearing para gene which carries mutations of the voltage- gate sodium channel (confering insecticide resistance) is not associated with infection but rather the ClipC9 gene directing the synthesis of Serine protease. This suggest that the effect of the resistant character on refractoriness to infection may be due to genes other than that involved in resistance to insecticides, and which happen to be linked to it. The Serine protease plays an important role in the activation of the three major immune signaling pathways in mosquitoes: Toll, Imd and JAK/STAT [20], which cause the release of antimicrobial peptides (AMPs) notably defensins, cecropins, attacin, gambicin and AgSTAT-A, effective against malaria parasites infections. Vontas et al. [63], using pyrethroid and organochlorine resistant An. gambiae strains, showed that defensin and cecropin are upregulated after pre-exposure to perme- thrin. This study sugggests that insecticide resistant mos- quitoes may be better equipped than susceptible ones to combat infections, but these two immune effectors alone may not be decisive in rendering the vector completely refractory to malaria infections as many other pathways activated concomitantly during parasitic invasion are altogether implicated in the outcome of a contamination [20].
In Culex pipiens which is vector of many pathogens including arboviruses [65], filarial worms [66], and protozoa [67], immune response was stimulated in an insecticide resistant field strain by injection of Lipopoly- sacharide (LPS) immune elicitor. As result, no difference was found in the expression of defensin and cecropin as compared to the control group; but only an increase in gambicin was recorded [68]. One point can be drawn from these results to infer what might happen in malaria vectors: Plasmodium infections may trigger the overex- presion of some immune factors while the other may have their expression either down regulated or unchanged.
The reactive oxygen species (ROS) produced by cellu- lar metabolism are another class of effectors of the innate immunity that can negatively affect malaria parasites [69, 70]. They kill the parasites through both lytic and melanization pathways [20]. Ingaham et al. [64] showed that An. coluzzii VK7 colony displaying kdr resistance mechanism, CYP6M6 and CYP6P3 metabolisers, had oxidoreductase overexpressed after sub-lethal exposure to deltamethrin, suggesting that this species could be more refractory to Plasmodium infection. At this point, it is necessary to verify whether under natural conditions, insecticide-resistant Anopheles mosquitoes will display an overexpression of ROS or not.
Cellular immune responses are carried out by varous type of hemocytes that eliminate pathogens by phago- cytosis, lysis and melanization [20]. Organochlorines and organophosphate were found to affect differently the hemocytes abundance including granulocytes in the insect Rhynocoris kumarii [71]. In mosquitoes, studies are needed to ascertain the impact of insecticide resist- ance on cellular immunity and the resulting effect on the infectivity of resistant vector to malaria parasite. Regard- ing melanization of pathogens, it is lead by the phe- noloxidase (PO) produced by Oenocytoids [72, 73] and is regulated by serine protease inhibitors. In field-caught C. pipiens resistant to insecticide through an increase in detoxification (esterase) and target site mutation (ace- 1), PO expression was equal to that of susceptible group [74], suggesting that some genes associated with immu- nity might not be affected by insecticide resistance char- acter in mosquitoes. No studies have verified the effect of insecticide resistance on PO in malaria vectors.
Impact of insecticide resistance on commensal intestinal microbiota of malaria vectors
Bacteria, fungi and viruses colonize the gut, salivary glands and reproductive organs of the mosquitoes. These microorganisms are mainly acquired from the environne- ment and its composition is largely influenced by its aquatic breeding sites [75, 76]. In addition, the microbi- ota composition is highly dynamic, varying greatly with localities and seasons [77–79]. These variations of micro- biota composition within field mosquitoes may partly explain the variability in infection levels in the field [80].
Mosquito microbiota has great potential for impeding the transmission of malaria by altering vectorial capac- ity [81]. Also, the microbiota is capable of influencing the biology of the host such as altering its immunity, nutri- tion, digestion, vectorial competence, reproduction, and insecticide resistance [82–87]. With the growing concerns about the rapid spread of insecticide resistance in Anopheles mosquitoes, some studies have explored the functions of the mosquito’s gut microbial communities in the development of resistance. For example, distinct microbita populations were found associated with organ- ochlorine resistance in An. arabiensis [86] and Anoph- eles albimanus [88]. Similarly, an association between specific microbiota and intense pyrethroid resistance was reported in An. gambiae [89] and Anopheles ste- phensi [90], suggesting a microbiota-mediated insecticide resistance mechanism. Dieme et al. [91] suggested that changes in the feeding behaviour of insecticide resistant vectors may lead to higher microbial diversity. This diver- sity could modify the repertoire of protective bacteria against pathogen infections and/or that of their enhanc- ers, with consequences on the vectorial competence [9, 92]. Recently, Bassene et al. [93] showed that, in the spe- cies An. gambiae and An. funestus, the microbiota was signifanctly different between P. falciparum-infected and non-infected samples, although the resistance status of these mosquitoes was not evaluated. More refined stud- ies are needed to characterize the microbial communities harboured by the insecticide resistant malaria vectors. Also the contribution of microbiota against other fac- tors to the vectorial competence of insecticide resistant malaria vectors remains to be investigated.
Impact of insecticide resistance on the longevity of malaria vectors
Mosquito longevity is a determinant factor for parasite maturation and could influences malaria transmission [94, 95]. In fact, the extrinsic incubation of Plasmodium in its hematophageous host is about 11–14 days. There- fore, only mosquitoes whose lifespan is long enough could allow the complete development of the malaria parasite to the sporozoite infective stage and participate in the transmission of the disease. With the emergence and spread of insecticide resistance [7], many investiga- tions were undertaken to gain knowledge of the effect of this phenomenon on the vectors’ longevity and so on its potential epidemiological impact.
So far, studies on the impact of insecticide resistance on malaria vectors longevity have focused on four spe- cies: An. gambiae, An. arabiensis, An. coluzzii and An. funestus. Globally, the findings revealed a pleitropic effect of insecticide resistance on mosquito lifespan [33, 96–108] (Table 2). The majority of studies (10/14) which used laboratory strains showed that pyrethroid resistant An. funestus and An. gambiae live longer than suscepti- ble ones [100, 106]. Of the studies including field strains, a longer life span was reported in organochlorine and pyrethroid resistant An. funestus strains [104, 105]. In contrast, a shorter life span was observed in An. gambiae strain resistant to organochlorine. It was reported that pre-exposure to insecticide in a manner micmicing field exposure to insecticides, affects the longevity of insecti- cide resistant An. gambiae strain [97], and that delayed mortality observed in the vectors may be dependent on resistance intensity [98]. This later observation indi- cates that findings obtained with laboratory colonies are to be taken with caution given that they may not reflect exactly what is oberved on the field [26]. Nevertheless, such studies remain important as they contribute to the understanding of the potential mechanisms affecting the vectors’ longevity [109, 110], notably resource-based trade-off and oxidative stress.
Study species | Origin of strains | Class of insecticides | Resistance mechanism(s) | Pre-Exposurea/Exposureb to insecticide | Effect on longevity | References |
An. gambiae s.l | Field | PY | Not available | Deltamethrin and permethrina | RR longevity > SS longevity | [97] |
Field | PY & OC | kdr | Not applied | RR longevity < SS longevity | [96] | |
Field | PY | Not available | Permethrin (Net) b | RRe longevity=RRne | [98] | |
Laboratory | PY & OC | kdr & P450 | Permethrin (Hut‐net) b | |||
Laboratory | PY | Not available | Deltamethrinb | RR longevity < SS longevity | [99] | |
Laboratory | PY | kdr, P450 and esterase | Not applied | RR longevity > SS longevity | [100] | |
Laboratory | OC | kdr | DDTa | RR longevity = SS longevity | [33] | |
CA | ace‐1 | Bendiocarba | RR longevity > SS longevity | |||
Laboratory | OC | kdr, GSTe, P450 & Es | Not applied | RR longevity = SS longevity | [101] | |
Laboratory | OC | GSTe, P450 & Es | Not applied | RRb longevity > RRs | [102] | |
Laboratory | OC | GSTe, P450, Es;kdr | Not applied | RR longevity < SS longevity | [103] | |
DDTa | RRe longevity = RRne | |||||
Permethrina | RRe longevity < RRne | |||||
Deltamethrina | RRe longevity < RRne | |||||
Malathion | RRe longevity < RRne | |||||
An. funestus | Field | OC | GSTe2 | Permethrin (Net) b | RR longevity > SS longevity | [104] |
Field | PY & OC | GSTe2 | Not applied | RR longevity > SS longevity | [105] | |
Laboratory | PY | Not available | Not applied | RR longevity > SS longevity | [106] | |
Laboratory | PY & CA | P450-a | Not applied | RR longevity = SS longevity | [107] | |
Laboratory | PY & CA | P450-a | Not applied | RR longevity = SS longevity | [108] | |
P450-b | RR longevity = SS longevity | |||||
P450-a/P450-b | RR longevity = SS longevity |
PY: Pyrethroid; OC: Organochlorine; CA: Carbamate; GSTe: Glutathion S-transferase; Es: Esterase; kdr: Knock down resistance; P450: Cytochrome P450 monoxygenase; RR: resistant strain, SS: susceptible strain; RRe: resistant strain exposed to insecticide; RRne: resistant strain non-exposed to insecticide; RRb: Resistant strain fed on blood; RRs: Resistant strain fed on sugar; > more; < less; = equal
Resource based trade-off is an evolutionary ecology concept that states that when environmental constraints lead to the augmentation of resources to one biological trait, other traits will have their energy budget reduced [111]. Accordingly, when mosquitoes adopt the detoxi- fication mechanism to prevent the effect of insecticide, an increased production of detoxifying enzymes follows and is maintained by the additional resources deployed for the function. Otali et al. [100] have demonstrated
that metabolism and longevity of insecticide resist- ant An. gambiae are lower than that of the susceptible strain. Moreover, they showed that the resistant strain has higher Reactive Oxygen Species (ROS), which are factors determining oxydative stress. In fact, the ROS are multifunctional molecules produced by cells of all organisms during normal metabolism [112, 113]. They have been pointed out as key aging factor in other organ- isms including Anopheles [114]. Therefore, mosquitoes that develop the capacity to cope with oxydative stress are likely to live longer. Oliver and Brooke [103] in an experiment evaluating the effect of oxidative stress on the longevity of both An. arabiensis and An. funestus bear- ing respectively kdr and Cytochrome P450 mechanisms demonstrated that these species live longer, and that Cytochrome P450 activity seems more protective against oxydative stress.
Rivero et al. [26] proposed the potential effect of dif- ferent detoxifying enzymes on vector longevity. For example, Glutathion S-Transferase is considered to pro- tect against oxydative stress. Confirming this point, a longer lifespan implicating Glutathion S-Tranferase in An. funestus was revealed with and without exposure to insecticide [104, 105]. In contrast, monoxygenase, known to be associated with an increase in oxydative stress has not led, as expected, to reduced longevity in An. funes- tus [108]. More studies using field populations and mic- micing field conditions are necessary to ascertain the full impact of insecticide resistance on longevity of the malaria vector.
Conclusion
The need for a comprehensive understanding of the impact of insecticide resistance on malaria vector com- petence is unquestionable. The current state of knowl- edge is not only insufficient but also contradictory to draw a definitive conclusion. A tendency nevertheless emerges from findings that insecticide resistance may increases the infectivity of malaria vectors to Plasmo- dium, thus their vector competence. This is possibly due to changes in the expression of some genes notably those involved in blood-feeding and the immunity. Addition- ally, microbiota communities vary in the resistant mos- quitoes as compared to the susceptible counterparts. The actual effect of these changes in the course of infection and their impact on the infectivity of malaria vectors to P. falciparum is still to be investigated. Finally, the longevity of the vectors is not always affected by insecticide resist- ance mechanisms. It is worth noting that, studies using vectors displaying metabolic resistance were under- represented because molecular markers to diagnose this character were developped only recently, especially in An. funestus. Malaria vectors that bear metabolic resistant mechanism are, on an ecological immunology point of view, expected to have a number of biological functions impaired, including immunity. If established, this situa- tion may cause them to become less refractory to Plas- modium infection. Taking advantage of recent advances in the genomics, transcriptomics and molecular charac- terization of insecticide resistance, more refined studies can now be undertaken to fill knowledge gaps regarding the effect of insectide resistance on key determinants of vectorial competence and subsequently predict changes in the epidemiology of malaria in a context of insecticide resistance escalation.
DDT Dichloro‐diphenyl‐trichloroethane GST Glutathione S‐transferases
kdr Knock down resistance
LPS Lipopolysacharide
PO Phenoloxidase
Vgsc Voltage gate sodium channel
Author contributions
PFS, EEN, MT, MMS, CN wrote the manuscript. TD and PFS prepared the figures and tables. CN and CW acquiered the funding. All the authors revised the manuscript. All authors read and approved the final manuscript.
Funding
The authors acknowledge the financial support from the Wellcome Trust, UK, through the International Intermediate Fellowship (220720/Z/20/Z) granted to Cyrille NDO.
Availability of data and materials
Not applicable.
Declarations
Ethics approval and consent to participate
Not applicable.
Consent for publication
Not applicable.
Competing interests
The authors declare no competing interests.
References
Accepted: 4 January 2023
1. WHO. A global brief on vector‐borne diseases. Geneva, World Health Organization; 2014.
2. Gelband H, Panosian CB, Arrow KJ. Saving lives, buying time: economics of malaria drugs in an age of resistance. 2004.
3. WHO. World malaria report 2021. Geneva, World Health Organization; 2021.
4. Phillips M, Burrows J, Manyando C. Malaria. Nat Rev Dis Primers. 2017;3:17050.
5. Cox FE. History of the discovery of the malaria parasites and their vec‐ tors. Parasit Vectors. 2010;3:5.
6. Sinka ME, Bangs MJ, Manguin S, Rubio‐Palis Y, Chareonviriyaphap T, Coetzee M, et al. A global map of dominant malaria vectors. Parasit Vec‐ tors. 2012;5:69.
7. Riveron JM, Tchouakui M, Mugenzi L, Menze BD, Chiang M‐C, Wondji CS. Insecticide resistance in malaria vectors: an update at a global scale. In Towards malaria elimination‐a leap forward. IntechOpen; 2018.
8. Elliott R, Ramakrishna V. Insecticide resistance in Anopheles gambiae Giles. Nature. 1956;177:532–3.
9. Sheldon BC, Verhulst S. Ecological immunology: costly parasite defences and trade‐offs in evolutionary ecology. Trends Ecol Evol. 1996;11:317–21.
10. Barnes KG, Weedall GD, Ndula M, Irving H, Mzihalowa T, Hemingway
J, et al. Genomic footprints of selective sweeps from metabolic resist‐ ance to pyrethroids in African malaria vectors are driven by scale up of insecticide‐based vector control. PLoS Genet. 2017;13: e1006539.
11. Donnelly MJ, Corbel V, Weetman D, Wilding CS, Williamson MS, Black WC. Does kdr genotype predict insecticide‐resistance phenotype in mosquitoes? Trends Parasitol. 2009;25:213–9.
12. Weill M, Lutfalla G, Mogensen K, Chandre F, Berthomieu A, Berticat C,
et al. Insecticide resistance in mosquito vectors. Nature. 2003;423:136–7.
13. Djogbénou L, Labbé P, Chandre F, Pasteur N, Weill M. Ace‐1 duplica‐ tion in Anopheles gambiae: a challenge for malaria control. Malar J. 2009;8:70.
14. Hemingway J, Hawkes NJ, McCarroll L, Ranson H. The molecular basis of insecticide resistance in mosquitoes. Insect Biochem Mol Biol. 2004;34:653–65.
15. Riveron JM, Irving H, Ndula M, Barnes KG, Ibrahim SS, Paine MJ, et al. Directionally selected cytochrome P450 alleles are driving the spread activity of midgut aminopeptidases. Arch Insect Biochem Physiol.
1990;15:149–63.
- Dana AN, Hong YS, Kern MK, Hillenmeyer ME, Harker BW, Lobo NF, et al. Gene expression patterns associated with blood‐feeding in the malaria mosquito Anopheles gambiae. BMC Genomics. 2005;6:5.
- Billingsley PF, Hecker H. Blood digestion in the mosquito, Anopheles stephensi Liston (Diptera: Culicidae): activity and distribution of trypsin, aminopeptidase, and alpha‐glucosidase in the midgut. J Med Entomol. 1991;28:865–71.
- Ribeiro JM. A catalogue of Anopheles gambiae transcripts significantly more or less expressed following a blood meal. Insect Biochem Mol Biol. 2003;33:865–82.
- Vijay S, Rawal R, Kadian K, Singh J, Adak T, Sharma A. Proteome‐wide analysis of Anopheles culicifacies mosquito midgut: new insights into the mechanism of refractoriness. BMC Genomics. 2018;19:337.
- Mitri C, Markianos K, Guelbeogo WM, Bischoff E, Gneme A, Eiglmeier K, et al. The kdr‐bearing haplotype and susceptibility to Plasmodium falciparum in Anopheles gambiae: genetic correlation and functional testing. Malar J. 2015;14:391.
- Vontas J, Blass C, Koutsos AC, David JP, Kafatos FC, Louis C, et al. Gene expression in insecticide resistant and susceptible Anopheles gambiae strains constitutively or after insecticide exposure. Insect Mol Biol. 2005;14:509–21.
- Ingham VA, Brown F, Ranson H. Transcriptomic analysis reveals pronounced changes in gene expression due to sub‐lethal pyrethroid exposure and ageing in insecticide resistance Anopheles coluzzii. BMC Genomics. 2021;22:337.
- Hamer GL, Kitron UD, Brawn JD, Loss SR, Ruiz MO, Goldberg TL, et al. Culex pipiens (Diptera: Culicidae): a bridge vector of West Nile virus to humans. J Med Entomol. 2008;45:125–8.
- Morchón R, Bargues MD, Latorre JM, Melero‐Alcíbar R, Pou‐Barreto C, Mas‐Coma S, et al. Haplotype H1 of Culex pipiens implicated as natural vector of Dirofilaria immitis in an endemic area of Western Spain. Vector Borne Zoonotic Dis. 2007;7:653–8.
- Kimura M, Darbro JM, Harrington LC. Avian malaria parasites share congeneric mosquito vectors. J Parasitol. 2010;96:144–51.
- Vézilier J, Nicot A, Lorgeril J, Gandon S, Rivero A. The impact of insecti‐ cide resistance on Culex pipiens immunity. Evol Appl. 2013;6:497–509.
- Molina‐Cruz A, DeJong RJ, Charles B, Gupta L, Kumar S, Jaramillo‐ Gutierrez G, et al. Reactive oxygen species modulate Anopheles gambiae immunity against bacteria and Plasmodium. J Biol Chem. 2008;283:3217–23.
- Kumar S, Christophides GK, Cantera R, Charles B, Han YS, Meister
S, et al. The role of reactive oxygen species on Plasmodium mel‐ anotic encapsulation in Anopheles gambiae. Proc Natl Acad Sci USA. 2003;100:14139–44. - James RR, Xu J. Mechanisms by which pesticides affect insect immu‐ nity. J Invertebr Pathol. 2012;109:175–82.
- Hillyer JF, Strand MR. Mosquito hemocyte‐mediated immune responses. Curr Opin Insect Sci. 2014;3:14–21.
- Lavine MD, Strand MR. Insect hemocytes and their role in immunity. Insect Biochem Mol Biol. 2002;32:1295–309.
- Cornet S, Gandon S, Rivero A. Patterns of phenoloxidase activity in insecticide resistant and susceptible mosquitoes differ between labora‐ tory‐selected and wild‐caught individuals. Parasit Vectors. 2013;6:315.
- Wang Y, Gilbreath TM 3rd, Kukutla P, Yan G, Xu J. Dynamic gut microbi‐ ome across life history of the malaria mosquito Anopheles gambiae in Kenya. PLoS ONE. 2011;6: e24767.
- Gimonneau G, Tchioffo MT, Abate L, Boissière A, Awono‐Ambéné PH, Nsango SE, et al. Composition of Anopheles coluzzii and Anopheles gambiae microbiota from larval to adult stages. Infect Genet Evol. 2014;28:715–24.
- Akorli J, Gendrin M, Pels NA, Yeboah‐Manu D, Christophides GK, Wilson MD. Seasonality and locality affect the diversity of Anopheles gambiae and Anopheles coluzzii midgut microbiota from Ghana. PLoS ONE. 2016;11: e0157529.
- Krajacich BJ, Huestis DL, Dao A, Yaro AS, Diallo M, Krishna A, Xu J, Lehmann T. Investigation of the seasonal microbiome of Anopheles coluzzii mosquitoes in Mali. PLoS ONE. 2018;13: e0194899.
- Sandeu MM, Maffo CGT, Dada N, Njiokou F, Hughes GL, Wondji CS. Seasonal variation of microbiota composition in Anopheles gambiae
and Anopheles coluzzii in two different eco‐geographical localities in
Cameroon. Med Vet Entomol. 2022;36:269–82.
80. Rosenberg R. Malaria: some considerations regarding parasite produc‐
tivity. Trends Parasitol. 2008;24:487–91.
81. Cansado‐Utrilla C, Zhao SY, McCall PJ, Coon KL, Hughes GL. The micro‐
biome and mosquito vectorial capacity: rich potential for discovery and
translation. Microbiome. 2021;9:111.
82. Rodgers FH, Gendrin M, Wyer CAS, Christophides GK. Microbiota‐
induced peritrophic matrix regulates midgut homeostasis and prevents systemic infection of malaria vector mosquitoes. PLoS Pathog. 2017;13: e1006391.
83. Song X, Wang M, Dong L, Zhu H, Wang J. PGRP‐LD mediates A. stephensi vector competency by regulating homeostasis of microbiota‐induced peritrophic matrix synthesis. PLoS Pathog. 2018;14:e1006899.
84. Romoli O, Gendrin M. The tripartite interactions between the mosquito, its microbiota and Plasmodium. Parasit Vectors. 2018;11:200.
85. Barletta ABF, Trisnadi N, Ramirez JL, Barillas‐Mury C. Mosquito midgut prostaglandin release establishes systemic immune priming. iScience. 2019;19:54–62.
86. Barnard K, Jeanrenaud A, Brooke BD, Oliver SV. The contribution of gut bacteria to insecticide resistance and the life histories of the major malaria vector Anopheles arabiensis (Diptera: Culicidae). Sci Rep. 2019;9:9117.
87. Martinson VG, Strand MR. Diet‐microbiota interactions alter mosquito development. Front Microbiol. 2021;12: 650743.
88. Dada N, Sheth M, Liebman K, Pinto J, Lenhart A. Whole metagenome sequencing reveals links between mosquito microbiota and insecticide resistance in malaria vectors. Sci Rep. 2018;8:2084.
89. Omoke D, Kipsum M, Otieno S, Esalimba E, Sheth M, Lenhart A, et al. Western Kenyan Anopheles gambiae showing intense permethrin resist‐ ance harbour distinct microbiota. Malar J. 2021;20:77.
90. Soltani A, Vatandoost H, Oshaghi MA, Enayati AA, Chavshin AR. The role of midgut symbiotic bacteria in resistance of Anopheles stephensi (Dip‐ tera: Culicidae) to organophosphate insecticides. Pathog Glob Health. 2017;111:289–96.
91. Dieme C, Rotureau B, Mitri C. Microbial pre‐exposure and vectorial competence of Anopheles mosquitoes. Front Cell Infect Microbiol. 2017;7:508.
92. Gabrieli P, Caccia S, Varotto‐Boccazzi I, Arnoldi I, Barbieri G, Comanda‐ tore F, et al. Mosquito trilogy: microbiota, immunity and pathogens, and their implications for the control of disease transmission. Front Microbiol. 2021;12: 630438.
93. Bassene H, Niang EHA, Fenollar F, Dipankar B, Doucouré S, Ali E, et al. 6S Metagenomic comparison of Plasmodium falciparum‐infected and noninfected Anopheles gambiae and Anopheles funestus microbiota from Senegal. Am J Trop Med Hyg. 2018;99:1489–98.
94. Garrett‐Jones C, Shidrawi GR. Malaria vectorial capacity of a population of Anopheles gambiae: an exercise in epidemiological entomology. Bull World Health Organ. 1969;40:531–45.
95. Ferguson HM, Maire N, Takken W, Lyimo IN, Briët O, Lindsay SW, Smith TA. Selection of mosquito life‐histories: a hidden weapon against malaria? Malar J. 2012;11:106.
96. Nkahe DL, Kopya E, Djiappi‐Tchamen B, Toussile W, Sonhafouo‐Chiana N, Kekeunou S, et al. Fitness cost of insecticide resistance on the life‐traits of a Anopheles coluzzii population from the city of Yaoundé, Cameroon. Wellcome Open Res. 2020;5:171.
97. Msangi G, Olotu MI, Mahande AM, Philbert A, Kweka EJ. The impact of insecticide pre‐exposure on longevity, feeding succes‐ sion, and egg batch size of wild Anopheles gambiae s.l. J Trop Med. 2020;2020:8017187.
98. Hughes A, Lissenden N, Viana M, Toé KH, Ranson H. Anopheles gambiae populations from Burkina Faso show minimal delayed mortality after exposure to insecticide‐treated nets. Parasit Vectors. 2020;13:17.
99. Viana M, Hughes A, Matthiopoulos J, Ranson H, Ferguson HM. Delayed mortality effects cut the malaria transmission potential of insecticide‐ resistant mosquitoes. Proc Natl Acad Sci USA. 2016;113:8975–80.
100. Otali D, Novak RJ, Wan W, Bu S, Moellering DR, De Luca M. Increased production of mitochondrial reactive oxygen species and reduced adult life span in an insecticide‐resistant strain of Anopheles gambiae. Bull Entomol Res. 2014;104:323–33.
- Oliver SV, Brooke BD. The effect of elevated temperatures on the life his‐ tory and insecticide resistance phenotype of the major malaria vector Anopheles arabiensis (Diptera: Culicidae). Malar J. 2017;16:73.
- Oliver SV, Brooke BD. The effect of multiple blood‐feeding on the lon‐ gevity and insecticide resistant phenotype in the major malaria vector Anopheles arabiensis (Diptera: Culicidae). Parasit Vectors. 2014;7:390.
- Oliver SV, Brooke BD. The role of oxidative stress in the longevity and insecticide resistance phenotype of the major malaria vectors Anoph- eles arabiensis and Anopheles funestus. PLoS ONE. 2016;11: e0151049.
- Tchakounte A, Tchouakui M, Mu‐Chun C, Tchapga W, Kopia E, Soh PT,
et al. Exposure to the insecticide‐treated bednet PermaNet 2.0 reduces the longevity of the wild African malaria vector Anopheles funestus but GSTe2‐resistant mosquitoes live longer. PLoS ONE. 2019;14:e0213949. - Tchouakui M, Riveron JM, Djonabaye D, Tchapga W, Irving H, Soh Takam P, et al. Fitness Costs of the glutathione S‐transferase epsilon 2 (L119F‐ GSTe2) mediated metabolic resistance to insecticides in the major African malaria vector Anopheles funestus. Genes (Basel). 2018;9:645.
- Okoye PN, Brooke BD, Hunt RH, Coetzee M. Relative developmental and reproductive fitness associated with pyrethroid resistance in the major southern African malaria vector, Anopheles funestus. Bull Entomol Res. 2007;97:599–605.
- Tchouakui M, Riveron Miranda J, Mugenzi LMJ, Djonabaye D, Wondji MJ, Tchoupo M, et al. Cytochrome P450 metabolic resistance (CYP6P9a) to pyrethroids imposes a fitness cost in the major African malaria vector Anopheles funestus. Heredity (Edinb). 2020;124:621–32.
- Tchouakui M, Mugenzi LMJ, Wondji MJ, Tchoupo M, Njiokou F, Wondji CS. Combined over‐expression of two cytochrome P450 genes exac‐ erbates the fitness cost of pyrethroid resistance in the major African malaria vector Anopheles funestus. Pestic Biochem Physiol. 2021;173: 104772.
- Remick D. Measuring the costs of reproduction. Trends Ecol Evol. 1992;7:42–5.
- Kirkwood TB, Rose MR. Evolution of senescence: late survival sacrificed for reproduction. Philos Trans R Soc Lond B Biol Sci. 1991;332:15–24.
- Garland T Jr. Trade‐offs. Curr Biol. 2014;24:R60–1.
- Rada B, Leto TL. Oxidative innate immune defenses by Nox/Duox family NADPH oxidases. Contrib Microbiol. 2008;15:164–87.
- Sumimoto H. Structure, regulation and evolution of Nox‐family NADPH oxidases that produce reactive oxygen species. FEBS J. 2008;275:3249–77.
- Monaghan P, Metcalfe NB, Torres R. Oxidative stress as a mediator of life history trade‐offs: mechanisms, measurements and interpretation. Ecol Lett. 2009;12:75–92.